The electromagnetic spectrum
Light (and all EM radiation) has two complementary properties.
We can describe it as waves of electricity and magnetism, or
as a stream of particles of essentially zero size containing fixed amounts of EM
energy.
Waves have physical extent; particles do not.
Particles carry fixed mass-energies; waves do not.
If we build an experiment to test the wavy properties of light,
we will find it is, indeed, composed of waves.
If we build an experiment to test the particle properties of light,
we will find it is, indeed, composed of particles.
We call the EM particles photons.
This fundamental wave-particle duality is the hallmark of quantum
mechanics.
All elementary 'particles' (electrons, quarks, photons, etc) are
both wavy and particly.
We will begin with the wavy nature.
Electromagnetic radiation is a "pure"
wave of electric and magnetic forces
which travels freely through the vacuum.
There is no underlying material which is waving.
If such a wave encounters a charged particle like an electron, the particle
will oscillate back and forth in response to the forces.
The wave is a solution of Maxwell's equations of electricity and
magnetism, perhaps the greatest physics accomplishment of the 19th
century.
The waves have five properties:
Direction of travel: EM radiation travels on a straight path in the vacuum.
Speed: EM radiation travels at 299,790 km/s in the vacuum for all
observers.
This speed of EM radiation is always represented with the letter symbol c.
Wavelength: the distance between repeating orientations of the electric force.
Polarization: the orientation of the electric oscillation,
always perpendicular to the travel direction.
Amplitude: the strength of the electric oscillation; the number of photons.
The spectrum of EM radiation refers to the variety of wavelengths possible.
All wavelengths are possible in a continuous progression from zero to infinity meters.
Since some of the wavelengths are very short, here are the metric system designations
for fractions of a meter:
cm: centimeter, 1/100 meter
mm: millimeter, 1/1000 meter
μm: micrometer or micron, 1/1000 mm = 10−6 meter
nm: nanometer, 1/1000 μm = 10−9 meter.
Another unit slowly going out of favor but often used by astronomers is the
Ångstrom (Å), equal to 1/10 nanometer.
The EM spectrum is divided into seven wavelength bands:
gamma-ray | X-ray | ultraviolet |
light | infrared | microwave | radio |
<0.01nm | 0.01nm-10nm | 10nm-400nm |
400nm-700nm | 0.7μm-1mm | 0.3mm-1cm | >1cm |
nuclear bomb | medicine | sunburn | vision |
"heat" radiation | ovens, comm. | communication |
The boundaries are kind of fuzzy.
Light is specifically defined as visible EM radiation, but
sometimes people refer to 'ultraviolet light' or 'infrared light'.
The visible spectrum is the familiar rainbow of colors produced by a prism
or a rainstorm:
400 nm =violet; 550 nm =green; 700 nm =red.

(4000 - 7000 Å)
Summary
Light is a form of electromagnetic radiation.
EM radiation can be described as oscillating waves of electric and magnetic
forces.
Different kinds of radiation have different wavelengths.
Blackbody radiation
All EM radiation is produced by vibrating charged particles; almost
all of the radiation astronomers analyze is produced by vibrating electrons.
Electrons are very common (all atoms have them), lightweight, and easy to set
into vibration.
What we perceive as heat or cold is just the vibrations of the particles
in a substance.
If those particles are vibrating faster than the ones in our finger nerves,
something feels hot. If they are vibrating slower it feels cold.
The average energy of motion of each particle in a warm object is about
kT, where k = 1.381×10−23 Joules/°K is
Boltzmann's constant (the Joule is the metric unit of energy) and the
temperature is measured in Kelvins.
The Kelvin temperature scale begins at 0° where particles have no vibrational
motion (so-called absolute zero), and uses Celsius divisions from there.
Water freezes at 273.15 °K and boils at 373.15 °K.
Electrons vibrating with energy E tend to emit photons with similar
energies.
The relation between the energy E of a photon and its wavelength λ
(Greek letter lambda) is E = hc/λ, where h =
6.626×10−34 Joule-sec is Planck's constant and
c is the speed of light (see above).
So, objects with temperature T tend to radiate photons with wavelength
λ ≈ hc/kT = 0.015/T meters.
Not all particles in an object vibrate with the same energy; some vibrate faster,
some slower, as they collide with each other and constantly exchange bits of energy.
The result is that a warm body emits photons with a wide distribution of
wavelengths.
Below is a plot of the energy flux leaving a hot, opaque
object of uniform temperature as a function of wavelength.
The quantity plotted is the number of Joules of radiation energy leaving each square meter
of the object each second in each nanometer band of the spectrum.
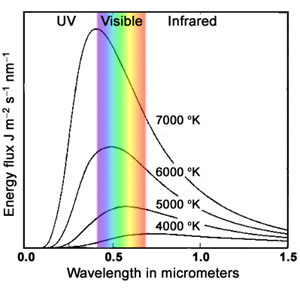
Notice that:
a) Increasing the temperature increases the brightness rapidly.
The total radiation coming from a hot body is proportional to T 4.
b) The most luminous wavelength band, like the average wavelength, is proportional
to 1/T: λmax (in microns) = 3000/T.
c) The apparent color of hot bodies changes from reddish at low temperatures
(more 700 nm than 400 nm photons), to white (more-or-less flat across the visible
spectrum), to bluish (more 400 nm than 700 nm photons) at high temperatures.
These distributions are called Planck or blackbody distributions.
Max Planck figured out why hot bodies radiate this way at the end of the 1800s
and presented his paper on 14 December 1900.
The reason is related to the particle nature of light, and Planck's work ushered
in an entirely new way of looking at the world, quantum mechanics.
The term blackbody refers to a perfect absorber, an object that absorbs
all radiation falling on it and converts the energy into heat before re-radiating it.
No exposed object is a perfect blackbody (the interior of a uniformly heated body
does contain this distribution of radiation).
However, many objects closely approximate blackbodies, including the Sun, most
other stars, rocks like the Moon and asteroids, incandescent lightbulbs, and
the human body.
We humans have a temperature about 300 °K and we radiate profusely at about
10 μm.
Below is a graph showing the spectral distribution of the Solar irradiance
at the top of the Earth's atmosphere in Watts (=Joules/sec) per square meter per
nanometer.
Data from The 1985 Wehrli Standard Extraterrestrial Solar Irradiance
Spectrum at
http://rredc.nrel.gov/solar/standards/am0/
The overall distribution of radiation is more-or-less like that of a blackbody,
but there are significant departures especially in the violet and ultraviolet
parths of the spectrum.
These departures introduce us (and the physicists of the 19th century)
to the next section.
Summary
Hot, opaque, objects like stars and planets radiate a continuous range of
wavelengths with most of the radiation coming out near
λmax (in microns) = 3000/T.
This radiation is produced by electrons vibrating with similar energies.
Atomic physics and spectroscopy
As early as 1814, the German optician Fraunhofer cataloged 754 dark features in
the solar spectrum.
The title square at the top of the page is a recent picture.
These "missing wavelengths" are signature absorptions by atoms in the
Sun's atmosphere.
Electrons in atoms
Students should be familiar with the general structure of atoms.
At the center of an atom is the nucleus, composed of roughly equal
numbers of protons (+1 charge unit) and neutrons (0 charge unit).
Almost all of the mass of an atom is in the nucleus.
Protons and neutrons have roughly equal mass, both being about 1830× the
electron mass.
A charge-neutral atom has as many electrons oscillating about the nucleus as
there are protons in the nucleus.
The cloud of electrons determines the chemical properties of the atom.
Each chemical element is then distinguished by the proton number,
called the atomic number Z, of the nucleus.
There are 92 naturally occuring elements, hydrogen through uranium.
The elements have periodic chemical properties.
For example, beryllium (Z=4), magnesium (Z=12), and calcium (Z=20) all
behave similarly.
The periodic table of the elements reflects this behavior.
The great achievement of quantum mechanics was the explanation of both the
periodic behavior and the dark features in the spectrum.
Most of us are taught in grade school that the electrons in an atom
orbit the nucleus somewhat like the way planets orbit the sun.
This picture is not very accurate.
Electrons, like photons, are waves when they are not being particles.
How are we to understand a wavy electron?
Every particle has a wave function which describes a probablility
amplitude over all possible locations and at all times.
The likelyhood (or expectation value) for finding the 'particle'
at a particular location is the amplitude squared.
Because the positively charged nucleus pulls on the negative electron, the wave of the
electron is confined to the neighborhood of the nucleus.
Imagine this wave circling around and bouncing back and forth against this
confinement.
It will overlap on itself over and over again.
Unless the wave exactly overlaps crest on crest each time it comes back,
negative portions of the wave will eventually cancel positive portions.
Only those waves that meet the condition of perfect overlap will exist.
A better analogy than orbits are the allowed vibrations of a tightened string.
The patterns of allowed waves depend on a number of factors, including the
presence of other electrons.
However, we can make certain general statements about which wave patterns
are allowed.
Each pattern can be described by four quantum numbers:
n: | the principle number, which describes radial
properties of the wave.
It may have any integer value from 1 to ∞.
An electron's average distance away from the nucleus increases with increasing n.
|
l : | the azimuthal number,
which describes angular properties.
It may have any integer value from 0 to n −1.
An electron's average distance away from the nucleus also increases with increasing
l, although not as much as with n.
|
ml: | the magnetic
number, which describes orientation properties.
It may have any integer value from
−l to
+l.
|
ms: | the spin orientation number of the electron spin.
It may have a value −½ or +½.
|
The Pauli exclusion principle states that no two electrons in an atom can have
the same set of four quantum numbers.
From that principle we can build the periodic table of the elements.
In the table below, electrons fill the boxes starting close to the nucleus at n = 1.
At each n,
there can be two electrons with l = 0:
the two spin states in the single ml = 0
state.
There can be six electrons with l = 1:
the two spins for the three different ml
values −1, 0, and +1.
Element | | | n: |
1 |
2 |
3 |
| | Z |
l: |
0 |
0 |
1x1x |
0 |
1x1x |
xxx2xxx |
Hydrogen | H | 1 | |
 |  |  |  |  |  |
Helium | He | 2 | |
 |  |  |  |  |  |
Lithium | Li | 3 | |
 |  |  |  |  |  |
Beryllium | Be | 4 | |
 |  |  |  |  |  |
Boron | B | 5 | |
 |  |  |  |  |  |
Carbon | C | 6 | |
 |  |  |  |  |  |
Nitrogen | N | 7 | |
 |  |  |  |  |  |
Oxygen | O | 8 | |
 |  |  |  |  |  |
Fluorine | F | 9 | |
 |  |  |  |  |  |
Neon | Ne | 10 | |
 |  |  |  |  |  |
Sodium | Na | 11 | |
 |  |  |  |  |  |
Magnesium | Mg | 12 | |
 |  |  |  |  |  |
Aluminum | Al | 13 | |
 |  |  |  |  |  |
Silicon | Si | 14 | |
 |  |  |  |  |  |
Phosphorous | P | 15 | |
 |  |  |  |  |  |
Sulfur | S | 16 | |
 |  |  |  |  |  |
Chlorine | Cl | 17 | |
 |  |  |  |  |  |
Argon | A | 18 | |
 |  |  |  |  |  |
... |
The chemical properties do not depend very strongly on the value of n.
They do depend on the l
value of the outermost electron and the number of other electrons with the
same l.
Thus beryllium is similar to magnesium because both have two
l = 0 electrons on the outside.
Spectroscopy
The boxes above that are empty (with all values of n up to ∞)
represent quantum wave states that are possible but empty.
It is possible to take an electron from one of the occupied states
and cause it to vibrate in one of these other states.
There are two ways to do that.
First, if another electron collides with the atom,
it might kick the confined electron into the empty state.
Second, if a photon comes through the atom it can kick the electron
into the new state.
Remember that photons are also a wave of electricity and magnetism; the waving
forces stimulate the new vibration of the electron.
Not just any photon can interact with an atomic electron.
Each photon is an indivisible particle of energy; the atom has to accept all
or nothing.
We mentioned above that the energy of a photon is inversely related to its
wavelength λ: E = hc / λ.
Each available vibration state for an electron in an atom has a particular
energy.
Generally, states with higher quantum number n have higher energy.
An electron in a high n state is on average farther away from the
nucleus; it takes work to pull it away from the positive charge.
Only those photons with just the right energy (equivalently just the right
wavelength), equal to the energy change necessary to lift the electron exactly
into a new state, will be absorbed.
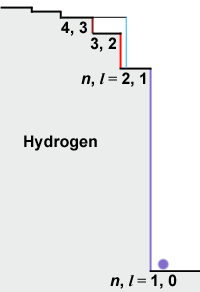 |
One way to picture the vibration states and their energies is like a staircase.
On the left we illustrate hydrogen, the simplest element.
The [n, l = 1, 0]
state has the lowest energy. To lift the electron up to the
[n, l = 2, 1] state
requires an ultraviolet photon of just the right energy, or the electron cannot
find itself on a "stair".
Once it is on an upper stair the electron can fall back again,
either by emitting a new photon,
or by giving the energy to another electron in a collision.
If the electron is in the
[n, l = 2, 1] state,
it is possible for a red photon of just the right energy to kick
it up one more step to the
[n, l = 3, 2] state.
It is these red photons which are "missing" in the spectrum of the Sun.
A blue-green photon can kick the
electron from [n, l = 2, 1]
directly up to [n, l = 4, 2].
In hydrogen, all the l values of
a given n value have the same energy.
To go from [n, l = 3, 2]
to [n, l = 4, 3] requires
a lower energy (longer wavelength) infrared photon.
|
Each different element has a different number of protons in the nucleus and
a different number of electrons.
Thus, for each element the "active" electron
(usually the outermost with the highest n and
l)
sees different forces and has different available vibration state energies.
The result is that each element absorbs a different set of photons.
For example, the faintish dark line in the yellow part of the Solar spectrum
results from sodium
[n, l = 3, 0] to
[n, l = 3, 1] absorptions.
At
this website
is a nice display of the emission spectra of various elements.
Emission occurs when the electron falls from an excited state to a lower
energy state closer to the nucleus.
Imagine the following simplified picture of a star.
Deep inside, there are enough vibration and motion states for electrons to
produce a blackbody distribution of electromagnetic radiation, independent
of the composition.
Near the surface, the gas is cooler and the atoms are less excited than
they are in the interior.
When the blackbody radiation shines out through the cooler layers, the atoms
there selectively absorb only those wavelengths which can excite their
electrons.
By measuring how much radiation is "missing" in each dark feature in the
spectrum, astronomers can measure the relative abundances of the various
elements in distant objects.
In other modules, we will see how we can measure velocities of
objects through the Doppler shift
of the wavelengths of an element's dark features in the spectrum.
Summary
The electrons in atoms follow very specific waves of probability, that only
exist at particular energies. Only one electron can occupy each wave
state.
The periodic table of elements is arranged according to which wave states are
filled with electrons.
If a photon with just the right energy (proportional to 1/λ)
comes along, it can kick an electron up into an empty state farther from
the nucleus. Each element has a different pattern of possible absorbing
photons.